Started in 2011, Neuro-Electronics Research Flanders (NERF) is doing research in what is an extremely competitive field: unraveling the architecture of the brain and nervous system. Its researchers want to reveal the anatomical and functional principles of neuronal circuits. But potentially even more impactful is the multidisciplinary approach and the research instruments coming out of this remarkable collaboration. Instruments for example that allow seeing the living brain at work and that will ultimately find an application in the diagnosis and treatment of brain disorders. Vincent Bonin, Karl Farrow and Alan Urban – three of NERF’s principal investigators – explain.
Scales matter
Vincent Bonin: “Karl and I are studying how sensory signals, especially those coming in through our eyes, are processed in the brain. Part of that is trying to find out how the visual cortex, the part of the brain that is responsible for visual information, is organized. But we also want to learn how visual signals spread activation over the whole brain and how that relates to behavior.”
“To do so, we need to look inside the brain, to see which brain cells are activated and what that activation causes further down in the brain. That is a very complex and time consuming task, especially because of the enormous difference in scales between the various structures we want to study: from the individual neuron measuring a few to a few tens of microns to activity in larger groups of hundreds or thousands of neurons. And from there, we want to look at patterns in brain areas with millions of cells and finally activity in the whole brain, which contains over 80 billion neurons.”
“But the instrument and tools developed to look at one scale, e.g. to monitor the activity of single neurons, cannot be used meaningfully at other scales, e.g. to see large patterns of activity in the whole brain. So we need more and better instruments to pick up small signals from many neurons in larger swaths of brain tissue.”
“That’s why the fact that we’re embedded in a major nanotech R&D center is so meaningful: here some of the instruments we need can be designed and built. "
"Think of the Neuropixels probe that is now deployed in labs worldwide and is able to simultaneously record the electrical activity of hundreds of neurons in a particular brain region. And another technique that we’re refining – functional ultrasound imaging (fUSi) – allows an even broader view into the activity patterns of the brain.”
Shining light on instinct
Karl Farrow: “I’m especially interested in innate behavior and how that ties in with the visual input. Innate behavior, we know, is generally driven by short-wired subcortical circuits. Circuits that are in the midbrain, the older part of the brain that we humans have in common with many organisms, deep below our big human, ‘thinking brains’.”
“The question now is: how does this actually work for visual stimuli? If, for example, a small animal sees a moving object above, it will immediately freeze or flee, apparently fearing a predator. But if we show the same moving object in front of the animal, it will remain calm and may even be curious to explore the object. Does that behavior relate to structures and connections in the brain? And how?”
“Visual signals come in through the retina, the light-sensitive layer of the eye. This is actually a fascinating and well-studied piece of tissue, in which we may distinguish over forty types of cells. And we can study fairly easy now which cells are activated by certain stimuli. The technique of choice is using animals for which specific cells in the retina are sensitive to laser light when they are activated. Then – very much simplified – we show the animals lines, blots, moving objects... while we simultaneously shine laser light on the retina and observe it through a microscope. The resulting fireworks show us which configurations of cells are activated for which input.”
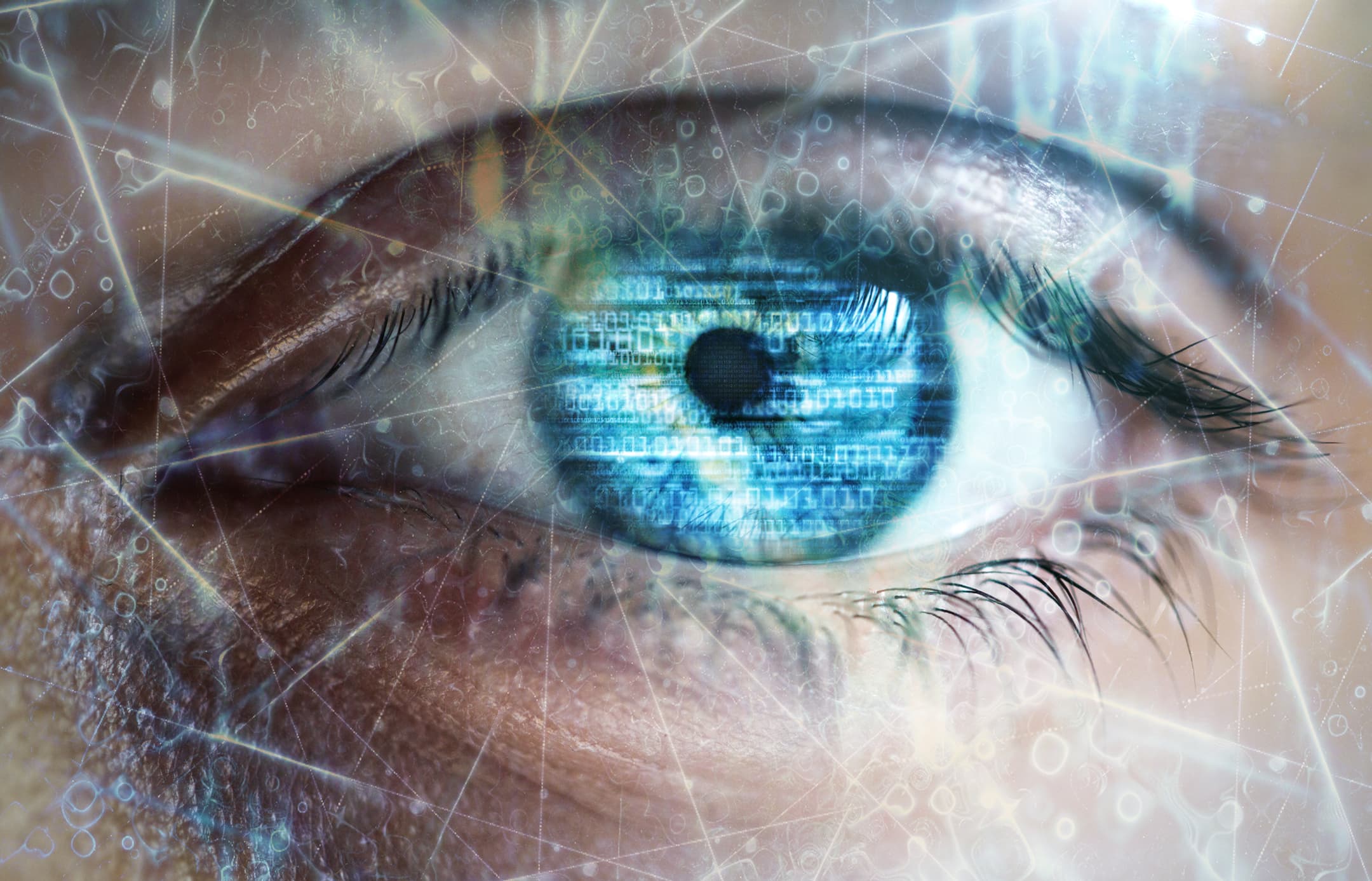
Visual signals come in through the retina, the light-sensitive layer of the eye. This tissue consists of over forty types of cells. Brain researchers (e.g. at NERF) are studying which cells are activated by certain stimuli to better understand how the brain deals with visual signals.
“From there on, innate activation goes to the so-called ‘superior colliculus’, a brain area that acts like a switchboard for signals between the retina and other brain regions. With our recent work, we’ve elicited some of these pathways, proving that the retina, superior colliculus, and deeper regions are not black boxes where every neuron is innervated by any other, but that there are indeed innate pathways. With cells in the superior colliculus who get preferential input from specific types of retina cells, and from there activate specific deeper regions.”
“In much of our work, we use imaging techniques that make single cell activation visible. But to trace activity from one cell to the other, we’ve used a variant where we inject a virus in the retina cells, a virus that is modified to emit fluorescent light and that can move from one neuron to the other so that we can follow the connections. That way, we were able to trace some of the connections to nearby brain regions.”
“But we can’t easily see the relation between the cells that are activated in the ‘superior colliculus’ switchboard and regions further down in the brain. That would require painstakingly following micrometer activity over millimeter to centimeter distances. And even more challenging is to get an idea of the simultaneous activation in the whole brain, which is what we’d really need if we want to relate vision and behavior."
See where the blood flows
Alan Urban: “Every neuron needs oxygen and nutrients to function, and both are brought along through an extremely dense network of very fine capillaries that serve every one of the brain’s billions of cells. As a result, there is a tight correspondence between local brain activity and blood flow, a phenomenon known as neurovascular coupling. So if we’d be able to monitor the variations of blood flow in each capillary, in real-time and in a non-invasive way, we’d have a tool to look at the active brain with an unprecedented field of view and resolution.”
“fMRI – functional magnetic resonance imaging – is one technique that exploits blood flow changes to make images of brain activity. Unfortunately, fMRI is hard to implement for work with small lab animals. It is also limited to experiments in anesthetized conditions and cannot be used to study the awake brain. Moreover, fMRI is expensive and has a somewhat limited resolution.”
“Another tool that’s used in medical practice for non-invasive, real-time blood flow monitoring is ultrasound imaging, where a focused ultrasound wave is emitted, reflected by the tissue, and recaptured for display. Moving elements like flowing blood in arteries, will cause a change in the reflected waves – the Doppler effect – that can be visualized. However, this original ultrasound technique can only be used for large arteries, not for the capillaries of the brain, and it cannot penetrate deeply enough to allow more than a superficial image.”
“fUSi – functional ultrasound imaging – doesn’t have these restrictions. It is a modified version of ultrasound imaging that instead of using a focused beam, works by emitting plain waves of high frequency ultrasound at a high frame rate through a 2D slice of the brain, receiving the reflections and then computationally reconstructing the image. By slightly shifting the ultrasound tool and making consecutive images, we get a 3D image of brain activity. fUSi was initially developed by Gabriel Montaldo, a senior scientist in my group.”
“Today, we’re still using traditional piezoelectric transducers to pick up the ultrasound signals. These are assembled from discrete elements and the number of sensors that can be added on a transducer is restricted by the cabling that’s necessary to read out the sensors’ data. A real step forward would be to replace the transducer with MUT technology (micromachined ultrasonic transducer), which consists of thousands of microsized drums carved out of silicon and which may have all readout electronics integrated. Once we’re at that level of integration, the resolution of the fUSi technique and the possibility to make 3D or even 4D images (including time), only depend on further scaling the electronics and the available computational power.
“Today, fUSi is already picking up speed as a lab tool and our multidisciplinary team is actively improving the technology. A few tens of labs worldwide are now using our recent developments to extend their research and better understand brain circuits. And with the scaling potential, fUSi could become a versatile, affordable instrument, part of the toolkit of any neurobiology lab.”
“But fUSi is not only suitable as a fundamental research tool. We also have the ambition to release this technology on the clinical market where it could be used during neurosurgery, e.g. when the surgeon is operating on the brain and wants to have a live 3D map of the active brain areas. And another application is with neonates, where fUSi can be used directly through the fontanelle or ‘soft spot’ at the front of a baby’s head. In some conditions, e.g. when an infant’s brain doesn’t receive enough oxygen and blood, doctors need to make rapid decisions to start treatments but they have no idea about the condition of the brain. fUSi is uniquely suited to help with these cases.”
Creating impact
Vincent Bonin: “The six NERF research groups form a unique ecosystem to test out new approaches to investigate the structure and function of brains. For our research we’re all developing and refining complementary techniques and tools. And because of our setup, it’s easy to collaborate – setting up effective collaborations within days, calling on someone’s expertise from another lab. That way, collectively, we’ve become an expert center for brain imaging. Recently, for example, we helped provide brain imaging for a paper on Alzheimer’s disease by one of the leading scientists in the field.”
“No less important is the collaboration with the nanotech R&D center imec in which we’re embedded. Imec’s engineers were instrumental in designing the Neuropixels probe. And they also have the expertise to eventually turn functional imaging into a powerful, versatile and compact tool.”
“We as NERF researchers want to have an impact on how brain science is done. That’s why we put so much effort in improving tools and methods that may change the way brain science is done. Making it much faster and more efficient, and thus opening space for more and new experiments. Experiments that will ultimately improve our understanding of the brain, how a healthy brain functions and what can go wrong when e.g. post-traumatic stress or Alzheimer’s disease strikes.”
Want to know more?
- Neuro-Electronics Research Flanders (NERF) is an interdisciplinary research center founded by imec, KU Leuven and VIB (Flanders biotechnology institute). NERF’s research aims to reveal anatomical and functional principles of neuronal circuits and derive new technologies for advancing the study of neural circuits, which will ultimately be applied in the diagnosis and treatment of central nervous system disorders.
- Recent study involving whole brain imaging with fUSi: https://www.nerf.be/news/whole-brain-imaging-of-mice-during-behavior
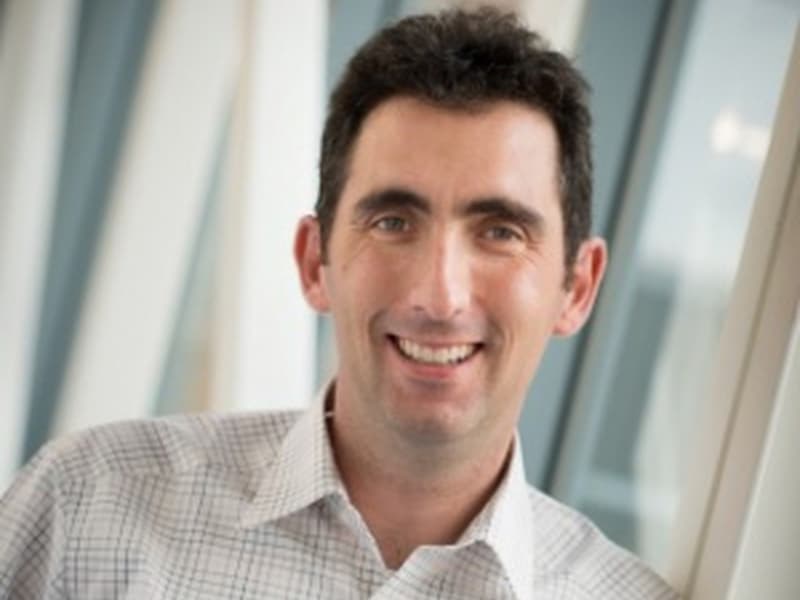
Vincent Bonin studied electrical engineering and computer science before turning to neuroscience. He did his PhD at ETH Zurich followed by a postdoc at Harvard Medical School. In 2011, Vincent moved to Leuven to start his own lab and help founding NERF. Vincent’s research is centered on the visual cortex, its circuitry and its contribution to sensory perception and behavior. The lab applies methods from biology, engineering and physics to elucidate mechanisms of sensory processing.
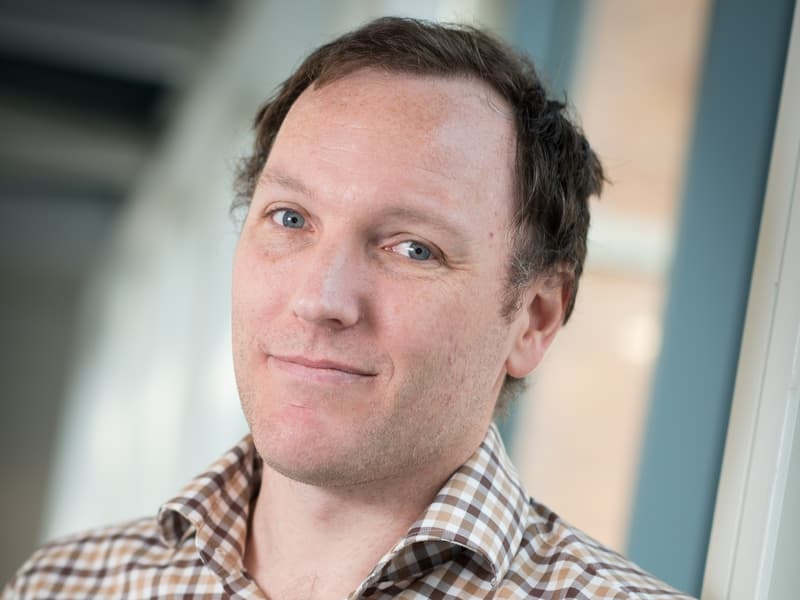
Karl Farrow pursued his doctoral research at the Max-Planck Institute of Neurobiology focused on dissecting small neural circuits in the motion detection system of the blowfly visual system. As a postdoctoral fellow at Harvard Medical School and the Friedrich Miescher Institute, Karl investigated how local circuits in the mouse retina are organized to perform cell type specific computations. At NERF, Karl’s current research focuses on delineating multi-synaptic circuits that span brain regions, linking the first stages of visual processing in the retina with activation of behavior by subcortical brain structures.
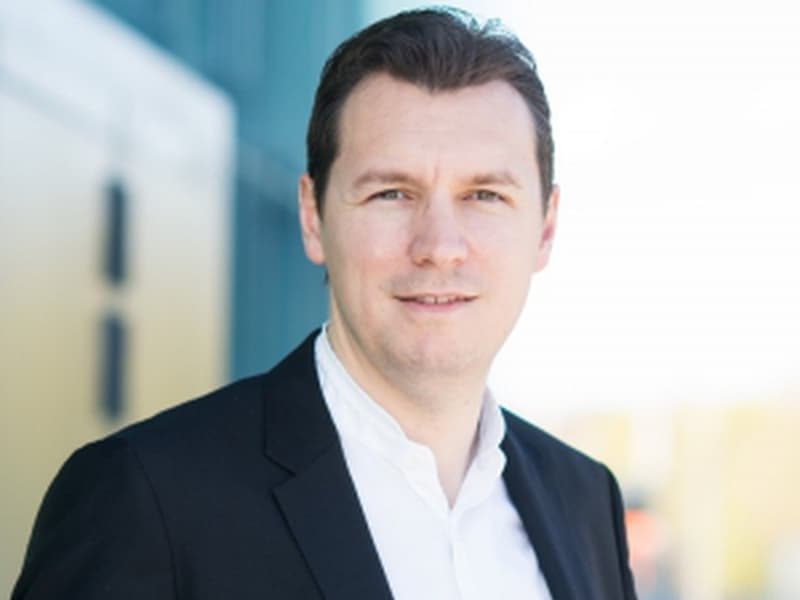
Alan Urban got his PhD in molecular and cellular biology from the Université de Lorraine, France. He graduated in experimental surgery from Paris Descartes university and was was assistant professor in neuroscience at the Ecole Supérieure de Physique et de Chimie Industrielle (ESPCI) in Paris. From 2012 to 2016, he led a research team at INSERM Hospital Sainte-Anne in Paris. Alan was also invited scientist at the RIKEN Institute (Japan) and in Tel-Aviv University (Israel). He created several companies and is an independent consultant in imaging technology. His team significantly contributed to the development of functional ultrasound imaging (fUSi). Since 2016, Alan Urban is a group leader at the Neuro-Electronics Research Flanders (NERF) and part time professor in the neurophysiology department at the KU Leuven.
Published on:
28 February 2019